The Magic of How Steel Works
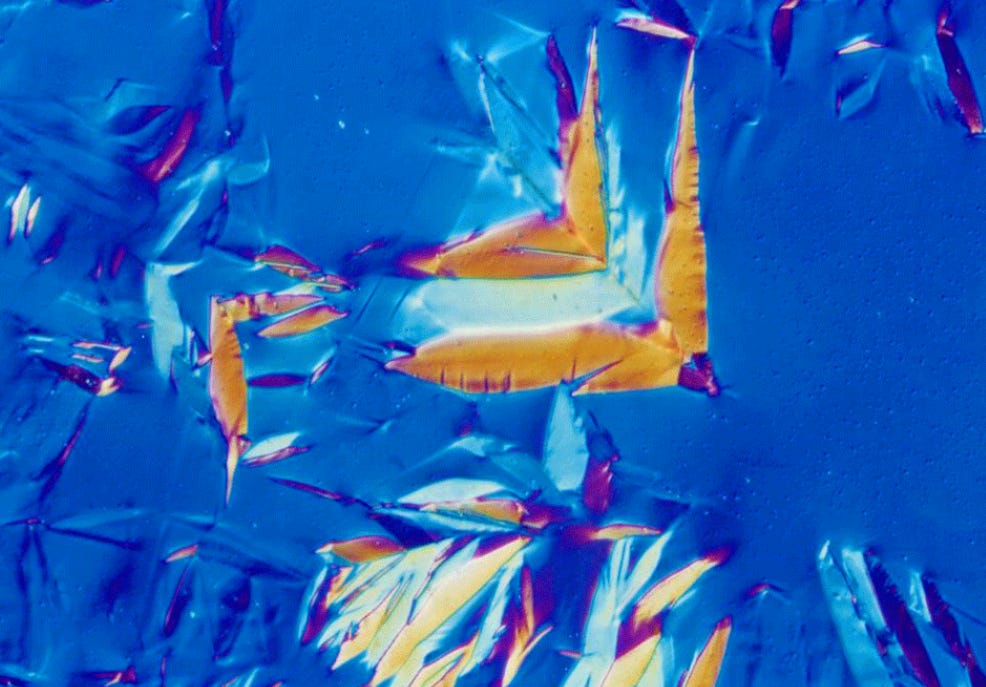
A lot of people have a strong intuition about how a lot of technologies work. When you look at cars and internal combustion engines, you sort of imagine how exploding gasoline drives pistons and makes rotational energy available to the wheels. When you think about computers, you might sort of understand that tiny bits of silicon can get turned from electricity blockers to electricity pipes, and this switching somehow enables computing using 1s and 0s, which are represented by the electricity being on or off at these tiny switches. Rockets, airplanes, vaccines, and video games all hold places in our brains that we at least imagine we sort of understand.
But very few people understand steel. At all.
We know it’s stiff and strong, but not usually brittle. It’s gray and shiny and doesn’t rust if we make it stainless steel, like our forks and knives. Japanese words use it in some sort of folded way.
But steel is more than a curiosity. It’s perhaps the strongest single technological basis for the modern world. Almost none of our technologies would be possible without steel. You need it to make rocket engines and jet plane rib cages. You need it to make cars safe and to hold semiconductors in place during high temperature manufacturing steps. Car engines are nearly impossible without at least some steel. Particle colliders, ferris wheels, ferrous wheels, oxygen tanks, and even the nuts and bolts that hold our machines together mostly require steel.
Iron Is Not Useful
Many steels are 90%+ iron, and some useful steels are over 99% iron. But 100% iron is not all that useful. It is much softer than steel, and not nearly as strong. It can be magnetized and drawn into wires, which is very useful. But there is one property that really hit me in the head when I was a child.
The front porch of my house was held up by not-very-decorative seven-foot tall structures of iron. These each were four bars of iron cast together at a midpoint such that the bars were spaced apart except at the midpoint of the pillar where a square held them together. This metal was probably somewhere between 100% and 99.5% iron. And it rusted on the top and the bottom, and we didn’t notice.
That is, until I was squatting down to turn on a hose. At that instant, the rust gave way and the probably 70-lb. pillar structure thing fell down and landed on the back of my head. I was astounded because it hit my head very hard. I pushed it off of me and stood up and felt my head and my hand came back bloody. My mom ran around the corner because she had herd the CLANG of those iron bars hitting my head. Turns out I was fine and didn’t even need stitches and I have no latsing sdei effetcs.
But it did leave an impression (thankfully not literal).
Looking back, I see why this unpainted iron structure landed on my head. Because iron never stops rusting. The oxygen that reacts with the surface of iron just keeps on diffusing into the metal rusting at deeper and deeper levels. Those beams rusted all the way through until they flaked away in a breeze and headed my way. Gold and platinum don’t hardly react at all with oxygen. Aluminum and silver will react, but the oxide that forms on their surface actually acts as an oxygen barrier, preventing deeper sections of the metals from oxidizing.
Our forerunners in the iron age saw this, and while using iron gave them major advantages, these shortcomings of iron (soft, quickly turns to rust, very heavy) held them back.
The Magic of Steel
Then someone figured out, probably by accident, that mixing ash into iron could change the world.
Ash is de-oxygenated carbon. Very fine, harshly-baked ash is almost pure carbon in many cases. And when you diffuse small little carbon atoms in among the much larger atoms of iron, then the iron stops being so soft. But why? Well, when it’s just iron atoms, they make a repeating (crystalline) structure like this:
The spacing between the iron atoms is fairly consistent in every direction. And because the electron configuration of iron ends in 4s², the outside electron shells of those iron atoms are actually largely like spherical balls. So because the balls are fairly equally spaced and their outside are actually spherical, it is fairly easy for those spheres to slip past each other in many different directions. So they do. This is why pure iron is so malleable and much softer than steel.
But then let’s add in some carbon.
Carbon is neat because it doesn’t just squeeze between the iron atoms, but it also bonds to the steel and itself. This makes it so that the iron atoms cannot move easily relative to each other. It is much easier to bend a small pure iron bar than a steel bar of the same size. The iron balls can’t move easily because the carbon is bonded to the iron and the carbon changes the spacing so it’s not so spherically symmetric, electron-shell-wise. Also, the carbon is sitting it neat little pockets in the corners between the iron atoms, and squeezing the carbon out of the pockets also takes energy. Energy that resists internal material slippage. This is all pretty fortuitous.
But there is more! The iron atoms have some fairly loosely-bound electrons near their outside, and those electrons are always ready to grab onto an oxygen atom to make a tiny piece of rust. But the carbon atoms in among the steel grab up all those weakly bound iron electrons and capture them into carbon-iron bonds. So in addition to the carbon making the iron not as soft, it also makes iron much more rust-resistant! Oxygen just sort of bounces off the steel atomic structure without bonding so easily to the iron. It still happens, but much, much more slowly.
But have you ever noticed that there are a lot of different types of steel? There’s the steel used in auto doors, steel used in auto engines, stainless steel, steel used for fences, steel used in dental things, and steel used to build buildings. There are dozens of different formulations of just carbon + iron steel, and they are good or bad for many different purposes. What makes them different from each other if they are all just made of two ingredients?
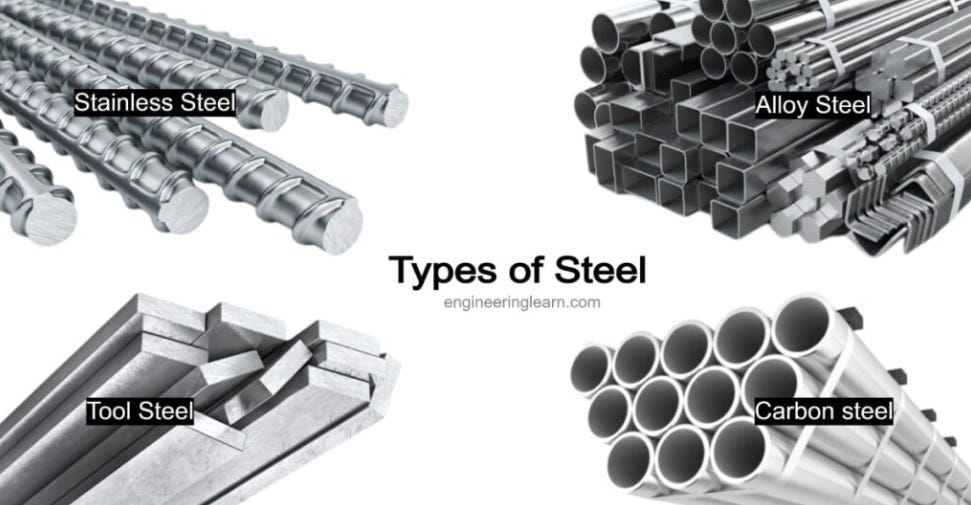
Well, there are two main differences, and they are related. One is the amount of carbon. If there is just a little bit of carbon, then only a few of the spaces between iron atoms contain carbon. Generally and up to a point, the less carbon, the softer the steel. The more carbon you add, the more brittle it becomes after a certain point. When there is too much carbon, then the crystal of iron becomes disrupted, and those disruptions are places where force can concentrate and start a crack or separation in the crystal.
But also, as you add more carbon, the whole crystal structure starts to find a different energy balance between the attractive and repulsive forces of the atoms involved. The packing structure of the atoms changes, and you actually get a different type of crystal. The three most common types of carbon-iron steel crystals are Face-Centered-Cubic (FCC), Body-Centered-Cubic (BCC), and Cementite. These different orientations only really talk about the distribution of the iron atoms and their spacing relative to each other. The carbon atoms just sort of fit in between the big iron atoms.
This is representative of the actual spacing, and cementite is not shown. The red and blue balls are all iron atoms. Here is how a carbon atom fits into this structure (the black ball):
Another way that material scientists like to display the three types of carbon steel are below, and this does include the more complex cementite. Again, all the balls are iron atoms…carbon is not shown at all (not even the black balls…those are iron atoms).
The way those iron atom-balls are packed together and how the carbon diffuses and adds its odd strength interact back and forth with each other. But those atomic configurations aren’t just controlled by how much carbon there is mixed into the iron.
The Beautiful Phase Diagram
You can change between the different configurations just by heating the iron-carbon mixture up. If you have 0.5% carbon mixed in by weight, you basically get FCC (austenite), usually denoted by gamma, “γ”. But then if you heat it up to 800C or higher, it all converts to BCC (ferrite), usually denoted by alpha, “α”.
If you go part of the way, say to 750C, then you get a mix of the FCC and BCC phase, a mixture of γ + α.
At different carbon concentrations, say 2% carbon, that is totally different. You still start with γ (FCC) at lower temperatures, but it all quickly converts to α (BCC) at 723C.
This matters because FCC steel and BCC steel have fairly different properties. They vary in terms of hardness, toughness, stretchiness, and even electrically, like how resistant they are to electrical current. Different applications require different types of steel.
Material scientists keep track of this complex triple-relationship of carbon concentration, heating temperature, and crystal types using something called a phase diagram.
Phase diagrams are an amazing tool for understanding all types of materials. Learning about phase diagrams 15 years ago really changed my life. I’ll explain why below. But first, let’s look in detail at the Iron-Carbon Phase diagram.
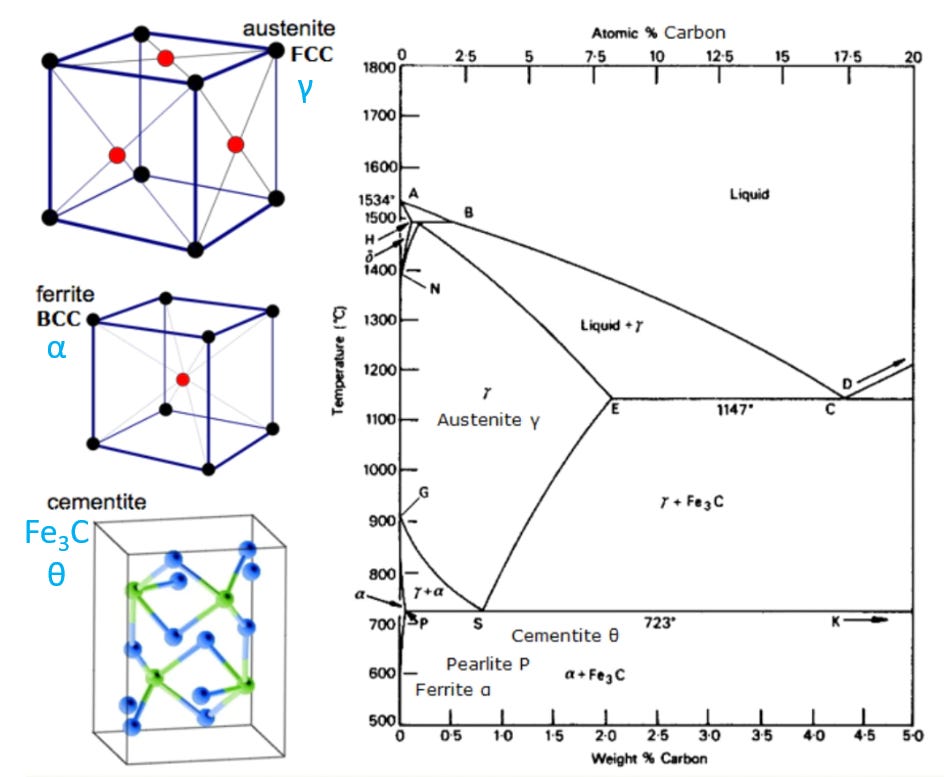
First, on the diagram below, you’ll notice the axes. This whole thing assumes you have a lump of steel with the carbon mixed in, and it’s cooled down from its molten state to below 500C. The Temperature vertical axis denotes what temperature you then are going to heat it up to and hold at for processing. You might heat it up to only 650C, and not much happens. But if you heat it up to the liquid temperatures (1200-1400C and above), it starts to melt. That top line is called the liquidus line, and you generally want to stay below that. If your metal melts, it resets all the crystalline structure and you sort of have to start over back at much lower temperatures.
The horizontal axis denotes the weight percentage of carbon in the mixture. It starts at zero and ends at 5% in this zoomed-in phase diagram. As you add more carbon than that, the phase diagram is sort of boring. All the interesting stuff happens at less than 5% carbon by weight in carbon-iron mixtures.
Let’s start by looking at 1.5% carbon mixtures. That is the vertical red line shown below.
Let’s start near the bottom of the red line. We have a 1.5% carbon mixture in iron, and we start to heat it up. At first, it’s a combination of BCC ferrite, Pearlite, and Cementite. Pearlite is just a mixture of ferrite and cementite, meaning they sit next to and around each other in balls and stripes, but they don’t mix on the atomic level (you get little zones of each). But when you heat this metal up to 723C and hold it there a few hours, you change to having a fairly homogenous mixture of FCC (γ) and cementite (Fe₃C), in much tinier lumps. Let’s keep heating it up. When you pass about 970C, you see we pass another black line. If we heat the sample up and hold it at around 1000C, then it turns into 100% FCC austinite (γ). A lot of steel processing has this as the goal. Austenite is a useful and strong crystalline phase of steel. But you better keep that sample below 1260C. If you go above that black line, the sample starts to melt, in little pockets of molten steel. You still have a lot of solid γ, but the higher you go, the more of the sample turns into liquid. Once you get above the black solidus line at around 1440C, it’s all melted. You lose all the crystalline structure and you have molten steel.
The Magic of Phase Lock-In
One super important thing about the carbon-steel system is that once you get to 1000C, and all your sample turns to γ, you can cool it down quickly, and it locks the crystalline structure in! Meaning, you can engineer the steel to be of different phases just by taking it up to high temperatures for a while and then cooling it down at a prescribed rate.
This capability has absolutely changed the world. Blacksmiths and sword masters have been doing it for many centuries, but when humans invented phase diagrams around 200 years ago, it unlocked a huge, systematic, repeatable way for humans to make steels. And starting around 1900, the scientific principles behind them came to light, enabling their theoretical, not just experimental exploration. Phase diagrams enabled the Industrial Revolution.
You can follow that phase diagram vertical journey for any percentage of carbon on your own.
If you do that journey at very low % of carbon (less than 0.5%), you’ll see that the melting starts at higher and higher temperatures, and if you stop and hold the temperature just below the melting point (1500C-ish), then you get the ability to create other crystalline forms of iron, like H and δ. You have to quench them fast to lock them in. That’s why blacksmiths have a barrel of water handy.
There are so many other types of phase diagrams for different metal combinations. There is copper-silver, and the very important-to-history copper-tin diagram, which is how we get bronze.
And if that looks complicated (it is!), then look at phase diagrams for ceramics. These involve more materials and are actually multi-dimensional, but they are often depicted in a triangular fashion for 2D reference use.
In this triangular diagram, the temperature needed to sinter (form) the different crystalline states is indicated by color. As you pick a point between the triangle corners, you are picking different ratios of the three ceramics involved.
This is how geologists determine how hot a rock sample got during its history, by reverse engineering the known phase diagrams of relevant ceramic mixtures. It’s incredibly useful for mining and oil exploration.
Understanding phase diagrams significantly enriched my life. They opened the door to my understanding of the relationship of chemical ingredients and the impacts on their material properties based on the heat they endure.
I have used this knowledge almost every week of my professional life for the last decade and a half, because I work on extreme, harsh sensing and evaluation solutions. Important and dangerous failures occur when materials turn into other things.
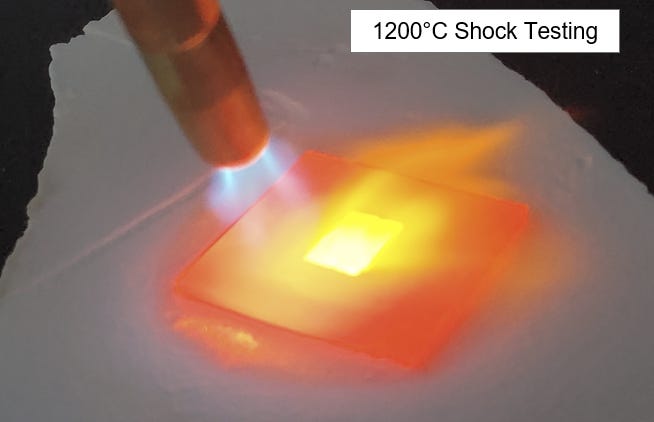
For instance, I once thought about making a ceramic pieces out of zirconia. But when I looked at the phase diagram of zirconia, I learned that a new phase happens at around 600C. My application was at 800C. Not a big deal, right? But that new phase of zirconia had a more expanded crystal structure. It made the zirconia take up almost 20% more space, growing 20% in volume! If I had used zirconia in my application, it would have broken apart due to internal stress fractures from that growth.
Now engineers ch0ose metals wisely based on their phase stability over their intended use range. When does that rust phase start to happen? In the early 1900s, several ships sunk due to steel having a phase change at -40C, to a phase that is much more brittle. The ships literally cracked and sunk as they traversed the arctic when they hit things that normally wouldn’t bother the ship hull metal at all.
Some people say we are entering a new Golden Age of Materials Science. In my opinion, the last 250 years and the next 250 years are a continuous, accelerating, awesome Golden Age of Materials Science.
And now you can more deeply appreciate that.