Asteroid mining is exciting and depressing at the same time. As amazing as the promise of 10 metric tons of platinum returned to Earth might be, most calculations show that the cost of retrieval using today’s technology would make this metal 10-100 times more expensive than just mining the metals at home. This is true even considering only near Earth asteroids and the tremendous recent progress in reducing the cost of taking mass to orbit.
The problem is delta-V (“delta” is change, V is speed in the right direction). If we want to mine an asteroid, we have to catch up with it, and then depart from it and go back toward Earth or Earth orbit. The difference in speed between Earth and most asteroids is tremendous — usually around 8,000 meters per second. If we want to send a craft to do anything but annihilate an asteroid (and itself), we have to accelerate to at least this speed differential. If we want to do so in one human lifetime, we also usually have to go faster than this and then also spend energy slowing down once we get their for a stable orbit or a soft landing.
Then we have to have brought along enough extra fuel to not only accelerate again to that speed to return to near Earth, but also enough to accelerate whatever mass is collected from the asteroid. No rocket ever created by humans has even 1/3rd the energy to attempt this and bring back marketable amounts of metal for even the most optimistic near Earth asteroid. The total delta V just to return a few hundred pounds would be on the order of 14,000 m/s, and the cost of even that delta V goes up with almost the square of the payload size. The more ore, the more fuel it takes to accelerate that ore back toward Earth. If this $500 million mission would yield around 5,000 ounces of material, the cost would be $100,000 per ounce. The most expensive metal, rhodium, tops out currently at around $10,000 per ounce.1
But we can build rockets that will put a decent amount of mass in orbit around the Moon. From Low Earth Orbit (LEO) to Low Lunar Orbit (LLO) only requires a total change in velocity of 4,100 meters per second.
What good does this do us? We want to mine asteroids, not the lunar surface. Well, I happen to have a good map of asteroids on the moon:
It turns out humans have been staring up into the biggest Treasure Map in human history for…all of human history.
For the largest craters, the asteroids and their metal content has been pulverized and sprayed widely. For the smallest craters, the rock would also likely be obliterated and scattered widely. But medium sized craters perhaps 50 feet across might be in the pay-dirt Goldilocks zone. These car-sized meteorites might have left some of their mass, pulverized, near the surface and in the middle of these craters, or splashed up around the crater walls. If we were on the moon, we might be able to go and collect meteorite-rich lunar soil.
But we are not on the Moon, and even if we were, the metal is at the bottom of the lunar gravity well. You have to gain 2,300 meters per second or so in velocity just to get into decent lunar orbit. This means sending a rocket, landing it on the moon, loading it with asteroid chunks, and blasting off. Again, very expensive and pretty much not worth it.
So I came up with a possible way to get that mass off the Moon’s surface without going down the gravity well: lasers.
We can send a small fleet of perhaps 3 to 5 laser-equipped, solar-powered satellites to the Moon. We can use low powered lasers to help map exactly where the asteroid stuff likely rests very near the surface (top few inches), or to spectroscopically scan where the pulverized asteroid dust is densest. Then we can use extremely strong but brief laser pulses to heat up the asteroid-rich soil, just a few grams at a time, but blazingly hot and very unevenly.
You see, small pieces of asteroid, each the size of a small grain of sand, can be heated by the laser to temperatures as hot as the surface of the Sun in just tens of billionths of a second. The surface of each of these grains, heated dramatically and very briefly on one side, will evaporate and get turned into a white hot plasma. This effectively turns each grain into a rocket that uses some of its mass as the rocket fuel in a process called laser ablative acceleration.
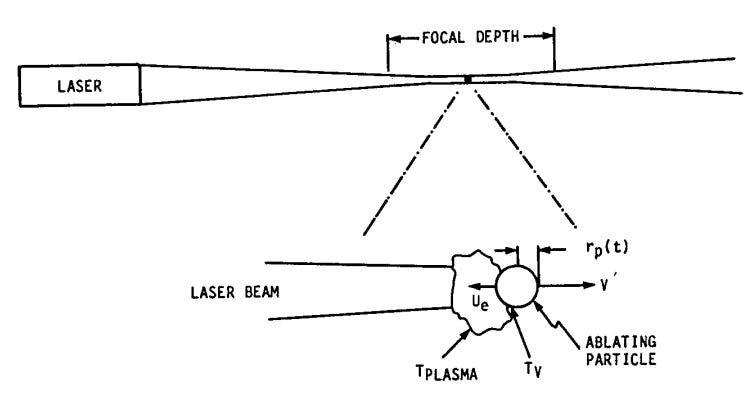
In a beautiful paper2 from 1986, Goela and Green describe how a single laser pulse of only 50 billionths of a second can accelerate a particle to speeds of over 3,000 meters per second. This is way more than is needed to put these particles into orbit around the Moon. The particle sizes studied were on the order of 50 microns in size, about the size of a small grain of sand or large piece of dust. Furthermore, to reach these particle speeds at these pulse times, less than 3% of the particle had to be used up as propellant, meaning 97% of the mass is put into lunar orbit or beyond.
The material ablated by the laser has an extremely high velocity of ~10^7 cm/s. This gives a specific impulse (Isp) of over 10,000 seconds (Isp is a very important measure of the effectiveness of rocket propulsion). To put this in perspective, the Space Shuttle Main Engine, an extremely efficient chemical rocket engine, had an Isp of 452.3 seconds. The most efficient thrusters humans have ever used in space are ion thrusters, with an Isp of a few thousand.
In other words, a small portion of each particle of ore is laser heated away as its own rocket fuel. In order to do this, the amount of energy needs to be 100 billion watts per each square centimeter, but only for pulses of fifty billionths of a second. This imparts around 10 joules to each tiny particle, enough to accelerate the particle to these awesome speeds.
Imagine a satellite orbiting the Moon at a height of 100km, shining down these laser pulses onto a target on the surface of the Moon. Some of those particles are heated from reflected light, and others directly, but bouncing off the lunar surface. Generally, they get accelerated in the direction of the laser beam as it reflects at an acute angle off the surface:
By using a train of pulses, you now have a pulsed train of clouds of particles heading into an expanding elliptical orbit around the Moon, with some of them leaving the Moon altogether. This Orbital Spray is not very useful, so we will add in some Laser Shepherds.
Existing slightly further ahead in a similar orbit to the first ore-launching Driver Satellite, two other less powerful Shepherd Satellites use very low power lasers to evaluate the Orbital Spray via reflected laser light. Cloud density, velocity (via Doppler Effect), and spread over time are evaluated by illuminating the possible cloud areas with much weaker laser pulses.
Then these same satellites can shine more powerful lasers strategically into the clouds to shape them into a Rivulet. This Rivulet can then be accelerated in just the right direction to circularize its orbit just below 100km, perhaps at 95km. This keeps the vast majority of the ore away from the satellites, but passing under them at a reasonable speed differential of just a few hundred miles per hour. Further Laser Shepherding can be done on the Rivulet at each pass. Shepherd Satellites can control altitude by setting cloud velocities. Other orbital parameters of the clouds and the overall Rivulet shape can be adjusted by positioning the Shepherding Satellites in slightly different inclinations and positions (such as mean anomaly or argument of perigee.) The more types of control desired, the more satellites are needed. This could be optimized based on the economics of the satellite insertion and the types of collection satellites used to gather the Rivulet when it is needed.
A surprising amount of refining of the asteroid ore can be done inside the Rivulet by the Laser Shepherd satellites. Metals, silicates, and oxides act very differently at different wavelengths. The Rivulet can be analyzed spectroscopically to see its free metal content, which is likely to be the most valuable stuff. Then different colors, angles, and powers of laser light can be used to selectively separate the types of particles that are undesirable. One color of laser light will preferentially accelerate silicates to an unstable, lower orbit, leaving only free metals in the Rivulet. In this way, it might even be possible to separate out particles by density, size, or composition into parallel Rivulets at very slightly different orbits, or into different pulse trains in the same orbital Rivulet. Basically, a combination of optical and inertial sorting.
One very convenient aspect of this setup is that the satellites don’t necessarily need to carry a lot of fuel. They don’t need to change orbital positions, and electrically powered sets of gyroscopes can be used to change where they are looking and aiming.
But wait. How can a laser be strong enough to do this from 100km above the Moon’s surface, and even much further than 100km if you are aiming at a 30 degree angle? That might be as far as 200km!
Laser beams stay collimated (keep their pencil-like beam shape) over long distances, but not forever. Beams spreading out is called “divergence.” And divergence is a factor of beam quality and something called the Diffraction Limit. A laser typically has one color, and that color is set by its wavelength, the distance in space between a peak and trough of its electromagnetic energy.
The amount a beam spreads is limited by this equation below, known as the Diffraction Limit:
θ=2.44λ/D
where θ is the angle of spreading in radians (one radian = 57 degrees), λ is the wavelength of the laser light, and D is the size of the lens the laser comes out of (assuming the laser comes out of the entire lens in a Gaussian distribution).
If we use blue laser light at 0.0004mm wavelength, and the lens is 4 meters in diameter3, then the least amount of laser beam spreading we will see is 0.00025 milliradians. In real life, even the best lasers and lens will see spreading about 2 times the diffraction limit. Which means that from 200km away, the beam will see spreading out of about 10 centimeters. If we set the focus of the beam at 200km, then this means the smallest achievable spot size is around 10cm.
Can we make a laser strong enough? Remember, we need 100 billion watts per square cm, and a circle 10cm across contains around 80 square centimeters. Now, most of the energy will be in the middle half of the circle, so we can focus on the middle 40 square centimeters. But that still means we need a 4 trillion watt laser to do asteroid laser mining on the Moon.
A single satellite system could launch 17 metric tons of material from the lunar surface to Low Lunar Orbit each year.
But we only need pulses of 50 billionth of a second, which means only 200,000 joules per pulse. Currently, fiber lasers can reach 100,000 joules continuous use in multimode fiber arrays, and Lockheed Martin claims to have a 300,000 joule pulsed laser weapon that fits on a vehicle, and they are optimizing those to be put into fighter jet pods. So while this technology is not yet available off the shelf, such high laser powers are feasible to fit into a large or medium satellite form factors in the very near future, and possibly today.
Powering this laser to fire 500 times per orbit (each orbit takes about 2 hours at this altitude) would require the expenditure of around 250 megajoules of energy (69 kWh), taking into account losses. This would require about 200 square meters of solar panels, or four solar arrays 5x10 meters each, which is less than one tenth of what the International Space Station deploys.
Assuming only a 5% laser-to-particle kinetic energy transfer to successful Rivulet orbit, this means that a single satellite system could launch 4kg of asteroid to low lunar orbit every 2-hour orbit, using only solar power. That is over 17 metric tons of asteroid-rich dust per year.
Once the Rivulet is established, other craft can fly through it collecting dense clouds of shepherded metals. Alternatively, patches of the Rivulet could be diverted by the Laser Shepherds into trans-lunar orbit, circling between the Moon and the Earth, for collection near Low Earth Orbit (LEO).4
There are many heated debates about whether asteroids are completely obliterated upon almost all impacts5, and there are debates about whether the lunar topsoil varies significantly in metal content. But even if this ends up being mostly just lunar regolith, and not rich in valuable metals, there are very many things that can be done with this much mass made available in lunar orbit. Radiation shielding can be built. Oxygen can be harvested. Some types of orbital construction can be performed, all with just the lunar regolith. If this lunar-surface-to-orbit system be created, then we have the immense value of a significant amount of mass in Low Lunar Orbit.
All in all, what’s described here is an almost massless, zero delta-V asteroid mining solution. And failing that, it is still a way to almost limitlessly put regolith into lunar orbit and beyond.
There are many kinks to work out, but I believe the physics is sound. And it might be enough to help jump-start economical space manufacturing.
Ad Astra Per Lux.
================================================
Author’s note: Special thanks to Dr. Phil Metzger of the University of Central Florida, a granular physicist with many publications on acceleration of lunar regolith plumes, for informally answering many questions I had about this subject. Any mistakes in this article are purely my own. Dr. Metzger did not review or edit this article.
I recently wanted to make a 3400°F wireless sensor out of rhodium. But Noooooooooo….
“Ablative acceleration of small particles to high velocity by focused laser radiation”, Goela and Green, J. Opt. Soc. Am. B/Vol. 3, No. 1/January 1986
To put the size of the mirror in perspective, Hubble’s main mirror is 2.4 meters. 4 meters would be possible but a tight fit with current rocket fairings, but the next generation of super heavy lift rockets will easily be able to accommodate a satellite with a mirror this size. Blue Origin’s New Glenn for example has a payload capacity of 6.2m in diameter with a length of over 11.4m
If an aiming of collection mistake were made, the small particles would simply burn up in the earth’s atmosphere, though any satellites in their way would likely be disabled or even destroyed.
While many believe that the impactor is completely vaporized, due to the speeds that asteroids hit at, others believe that certain sizes of asteroids allow significant parts of the impactor to remain. The impactor remnants are pulverized and lodge in the crater walls. If the crater is large enough, over 12 miles, the remnants will collect in a central peak of the crater.