What will the energy grid look like in 2072, fifty years from now? Energy policy has become a political football, but here we will cut out all that noise out and focus on the core economic, scientific, and geographical parameters that will decide our energy future.
This is more than just an intellectual exercise. Anyone that has a clear view of what the energy future is will have business and investing advantages. A psychological benefit is that we can decouple ourselves from the less rational political fashion trends of energy policy. Maybe some of us can even help influence smarter policy some day.
If you want to have a useful and deep understanding of what matters for the future of global energy, you have to understand what matters and how to evaluate different technologies. I have over a dozen years as a researcher in the Energy Industry, so this will be fun.
There are core functions that modern energy grids must have:
Reliability - Consumers must always have as much electricity as they need, with on-times above 99.8%. Brownouts are unacceptable. Energy rationing is unacceptable. Restrictions on what can be used at your house or business at different times are mostly unacceptable. The grid should just work however people need it all the time.
Cost of Plants and Infrastructure - The basic cost of generating and transporting the energy cannot be too high. Costs of generation plants cannot be too high, and investment timelines cannot be too long before return. Electrical transmission equipment is currently already paid for, but it does require some maintenance costs. New infrastructure into existing town and city layouts is very expensive. This also includes energy storage.
Non-Polluting and Sustainable - This is somewhat subjective, but the things like coal are simply not acceptable because of the sulfur dioxide and heavy metals that directly cause respiratory issues1. Technologies that require immense use of rare materials also are not going to work out at scale. Carbon emission matter as well to some extent.
This article series will delve into the different known and possible technologies for generating, transporting, and storing power, and will evaluate them by the above criteria dispassionately and with a view toward each technologies best possible version, given known physics and likely economics.
I will also secretly train you to know many of the most important deep concepts for the power industry.
We will look first this week at power generation (converting energy in some form to electrical energy). One thing that will be clear is that reliability of intermittent energy sources is strongly affected by whether they can be stored. Where that storage is electrical storage or a solution that doesn’t require a specific energy plant connection, we will address those storage mechanism separately in the storage section. Where that storage is non-electrical and specific to that energy generation method, we will discuss the energy generation and storage together.
Hydropower In Rivers
This is usually a very reliable, cheap, and non-polluting source of energy. Even during droughts, most hydroelectric stations can keep running because the river was dammed in order to create a humongous (sometimes many square miles large) reservoir of water at high gravitational potential energy.
Hydropower is an excellent introduction to how turbines work. The middle of each turbine is a long, thick rod called a rotor. Attached to that rotor are blades. The tilt and length of those blades are optimized to maximally turn the passing of water (or steam or burned gas in non-hydropower turbines) into rotation. It’s sort of like the opposite of how a fan uses rotational energy to blow a fluid.
A generator with its own long central is bolted to the long turbine rod usually behind or in front of the turbine. When the turbine rotates, the generator rod attached to it also rotates. Usually that generator rod has permanent magnets and the spinning part inside the generator is surrounded by stationary coils all packed together.
When you spin magnets inside metallic loops, an electrical current and voltage is generated — electrical power. Thus, in many, many types of power plants, whether coal, nuclear, natural gas, or hydropower, fluid pressure energy is turned into rotational energy in a turbine, which is attached to a generator that turns that rotational energy into electrical energy.
Hydropower depends on the fact that the water wants to fall, and the turbine takes advantage of that moving water to make electrical power. Really simple.
But there are two big issues with hydropower:
a) Most dam-able rivers have already been dammed.
b) The environmental impacts of blocking and damming rivers is quite significant, especially to migratory fish. I expect that there will actually be a strong movement to un-dam rivers starting soon and to be carried out during this century. People will rally to return rivers to their pristine states until it becomes a widely-held consensus.
Prediction: Hydropower will not grow and will likely shrink in the next 50 years.
Tidal Power
This is a very untapped source of energy which is fun to frame as lunar power (as opposed to solar power). Unfortunately, tidal power is extremely diffuse, and harvesting it via underwater turbines is only feasible where tidal energy density is quite high. And even in those areas, most large underwater turbines are only on the megawatt scale.
Tides come in and out twice daily, and when at high or low tide, there is no movement of water and so no power generation. This means the hour-to-hour reliability is extremely poor, perhaps one of the poorest out of all the options we are evaluating. But coupled to electrical energy storage, this might become a viable energy source.
This is a really important concept for many of our options. If we can store the electrical energy, then it doesn’t matter much how intermittent or variable our energy source is. The problem is that storing electrical energy on the scale of a town’s power grid is a massive challenge. Even for relatively small 2 megawatt tidal power turbines, the batteries required to store even 3 hours worth of power to make up for the lulls associated with high and low tide. Energy storage can “cover a multitude of sins” as it were.
But even if we could solve the energy storage problem (much more on that later), there are three major things issues with tidal power:
a. As mentioned above, the energy density is low in most places, so only in coastal chokepoints such as the Bay of Fundy and small sections of northern Britain is tidal power even close to being feasible.
b. Large underwater turbines are a threat to medium and large sea life. We have almost no visibility into this, literally.
c. Operating and maintaining large underwater rotating equipment is very hard. Corrosion, growths (such as barnacles), and general difficulty of repair make maintaining such infrastructure quite frightful and expensive. Imagine diving to perform a weld repair in a coastal area where the tides are selected for high velocity.
Prediction: Tidal power will not see wide application at any point in the future.
Geothermal Power
I made a fascinating discovery a few months ago when I was trying to figure out whether ground thermal conduction cooling could be used to keep crypto miners from overheating. It turns out that soil and rock do not let a lot of heat pass through them. They are pretty good thermal insulators. The way you measure this is in how many Watts (Joules per second, or thermal power) can pass through a surface, given a certain temperature differential (heat “flows” from hot to cold). Thus the units that I find most useful are Watts per square meter (times length in meters of thickness) per degree Centigrade. If that value is 10 Watts/m x C, then if I have one square meter of a material with 25C on the cold side and 75C on the other side, then I get:
1 square meter x 50C differential x 10 W/m x C = 500 Watts of thermal energy
Through that surface. That’s 500 Joules of energy transfer every second. I found that for loose sand, that value is depressingly low, only about 0.7 W/m x C. There was no way that I could create a bitcoin enclosure that used spikes to conduct heat to the ground, as it would disperse only 1/10th the thermal power generated by even the most modest economical mining equipment.
In geothermal energy plants, we want to go the other direction. We want to have as much energy transfer as possible. Unfortunately, that value is still pretty low even for the most conductive types of rock, like wet granite. In those cases, we are only at 3.7W/m x C. What does this mean for energy flows?
Well, let’s imagine a set of large boreholes whose circumference is 1 meter. Thus, for every length of the borehole at the deepest, hottest parts underground, we have 1 square meter of area. Let’s assume that the hot part of a borehole is super long…1 kilometer. then you have 1000 square meters. Let’s then assume the hottest possible temperature in a stable drilled area. The hottest geothermal temperature ever used is 400C, in Campania, Italy. Now, let’s presume we have 100 of these holes drilled, which is an extremely expensive and large geothermal project. This means that we have 25,000 square meters, and the temperature differential between the ground and the cool part of the heat exchange system is about 350C. How does the math work out? We have:
3.7 W/(m x C) x 25,000 square meters x 350C total differential = 130 Megawatts.
This is a nice installation. Note that most stations have a temperature differential of 60-110C or so. Our super-high-temp example, though, is still only about 1/10th the size of a typical power plant; but you can always string them together. The largest geothermal site in the world is in California, and it produces up to 1,205MW.
But here is where the problem comes in. That California installation uses geothermal energy very close to the surface — its name is The Geyser Geothermal Complex. It also takes up 68 square kilometers. People are just not willing to endanger natural wonders like geyser anymore. Further, even in the best situations, close-to-the-surface geothermal takes up large amounts of space.
Also, there just aren’t that many areas on earth with accessible geothermal energy. You have to drill like crazy and at great expense to access geothermal power in the vast majority of areas.
Prediction: Geothermal works ok in some areas, but is uneconomical or impractical in most areas. The US has less than 4,000MW of geothermal power installed, and this will never be a significant contributor, staying at around 1% of national capacity and far less than that as a percentage of global energy production.
Nuclear Power (Uranium or Plutonium Fission)
Before we talk about this, I just have to show you a really cool 1 minute video I found showing nuclear reactors starting up, including what they look and sound like. It serves no purpose in this article except that I think it is really cool:
Nuclear power works great as a base load source. “Base load” is a term meaning the electricity demand that’s always there. Let’s say that we have a daily fluctuation in demand in Oklahoma of 500-800MW. That top, fluctuating 300MW in demand (or supply) is the variable power, while that bottom 500MW that is sort of always needed no mater what is considered base load.
Nuclear plants provide constant energy, as their source of power is simply radioactive decay, usually mediated by the water and graphite that is supplied around the core. That water and graphite slows down the radioactively-emitted neutrons that come from the Uranium fuel as it splits. Without the water and graphite to slow the neutrons down, the neutrons would be moving too fast to be “caught” by other uranium atoms. When a uranium atom catches a neutron, it splits, releasing a lot of energy and 2 or 3 neutrons.
So nuclear plants can control (with these control rods of graphite) what % of the neutrons are slowed down enough in order to be easily caught by the surrounding uranium atoms, and thus the control rods control how fast the nuclear reactions (and thus heat production) happens.
It seems like this could be a fast process, but it usually takes around 24 hours to take the a nuclear plant completely online or completely offline. Thus, nuclear plants are almost always used as “base load” parts of the energy grid.
Which sounds nice, but is one of the two big problems for nuclear going forward. Here are the two big problems with nuclear:
There is a lot of pressure socially and economically to add renewables, especially solar and wind to the energy mix. These are extremely variable sources, and so the rest of the energy production needs to be able to come online quickly (like in half an our so) in case it is suddenly cloudy or not windy. Nuclear plants cannot do this that quickly. Typically nuclear plants can only modulate their output by 30% in around 3-6 hours, and take a day or more to be turned completely off or on.
Nuclear Plants are SUPER-NIMBY2 and also regulatory nightmares. Even if you can find a place where a community is happy to have a nuclear plant these days, the permitting for a nuclear plant plus its build time is now projected to by on the order of 20 to 25 years. Imagine being an investor who will not see their first penny of revenue (not to mention profit!) back for 20 years. Who knows what the regulatory or energy situation will be like in 20 years! Without investment, there will be no new nuclear plants, at least not in the US.
The newest US commercial power nuclear reactor went operational in 2016. Before that, the newest one was in 1996. The average age of US nuclear power plants is 41 years old. Here is the total US nuclear capacity over time. It’s not growing.
Now, there is a of talk of breeder, thorium, and pebble reactors. People are excited by these new technologies. But they are not being built as commercial reactors in the US any time soon, at least not at reasonable scale. And if they were built to scale, it is very likely that they would be burdened with the same 20 year regulatory hurdle nightmare for every single new nuclear power plant.
Prediction: New nuclear is dead, at least in the US. It has been regulated out of business.
Coal
Coal is cheap, and a lot of coal plants exist. But it is nasty, pollution-wise. when coal was 40% or more of the US energy mix (up until about a decade ago), coal pollution was responsible for an estimated 8,000 deaths by respiratory illness per year.
This number has nothing to do with carbon dioxide, but rather sulfur dioxide and to a much lesser extent, heavy metals. Burning coal puts those things into the air, and people breathe it in, and they get sick and die. The coal industry has done a lot to clean it emissions, dropping them by 70% in the last two decades, but not all of it can be scrubbed.
This is a moral hazard, and a perfect example of an unpaid externality. An unpaid externality is a cost that society pays for a technology to exist and function that is not directly paid by the industry that uses it. Before the 1970’s, chemical factories might dump effluent into rivers and kill off most of the fish. This caused a major economic problem for the downstream fishing industry, but the chemical factory didn’t have to pay it. It was an unpaid externality.
Most governments recognize this issue with coal’s huge unpaid externality now, and they are mostly working to reduce the number of coal plants, especially in the United States. The unpaid externalities are catching up with coal.
Prediction: Coal is cheap in one way, but expensive in many other ways. Governments will phase it out, usually at their first reasonable opportunity. There won’t be many operational coal plants in most of the world 50 years from now.
Natural Gas
Natural gas plants have a lot of interesting advantages:
a. 1/3 less carbon dioxide emitted vs. coal, and basically none of the other pollutants like sulfur dioxide or heavy metals. Only a tiny amount of nitrous oxides and carbon monoxide is released, and this is very tightly regulated, at least in the U.S.
b. The plants get permitted and built fairly quickly, making investment very feasible because of a quick return (versus nuclear).
c. Small footprint per gigawatt. A gigawatt nuclear or coal plant will take up 40-100 acres. A gigawatt wind or solar plant will take up many thousands of acres. I gigawatt natural gas plant can take up as few as 10 acres.
Another huge advantage of natural gas plants is that they can be turned from zero output to full output in as little as 20 minutes. As mentioned before, this is HUGELY important as more renewables like solar and wind are added to the grid, with all their variability.
These natural gas plants used to run at nearly full capacity all the time, providing the base load of the grid, the non-variable part that is always needed. But now more and more of these are running as “peaker” plants. Peaker plants are brought online to help smooth the electrical supply of the grid in order to keep the grid supply matched to demand, even when the supply varies a lot or the demand varies a lot (you’d be surprised how much power demand peaks at 5:30pm during weekdays when everyone comes home from work).
Natural gas peaker plants are pretty much the only reason that so much wind and solar has been able to be added to the grid. Without these plants acting as supply smoothers, solar and wind would haver created way too much grid variability.
Natural gas plants have also benefitted from the fracking revolution, which in the last 15 years has made America the Saudi Arabia of natural gas, keeping prices historically low for a very long time.
Pretty much the only downside to natural gas is the natural gas. Over the life of a plant, 2/3 of the cost of the plant is the purchasing of fuel. And of course it does emit carbon dioxide, even if it is 1/3 as much as coal.
Prediction: Especially in America, natural gas will be a large part of the grid until massive amounts (many terawatt-hours) of grid storage is available to handle days’ worth of variability in renewables. We will likely see a moderate slow-down in new natural gas plants over the coming decades, but very few of them will be retired early.
Fusion Power
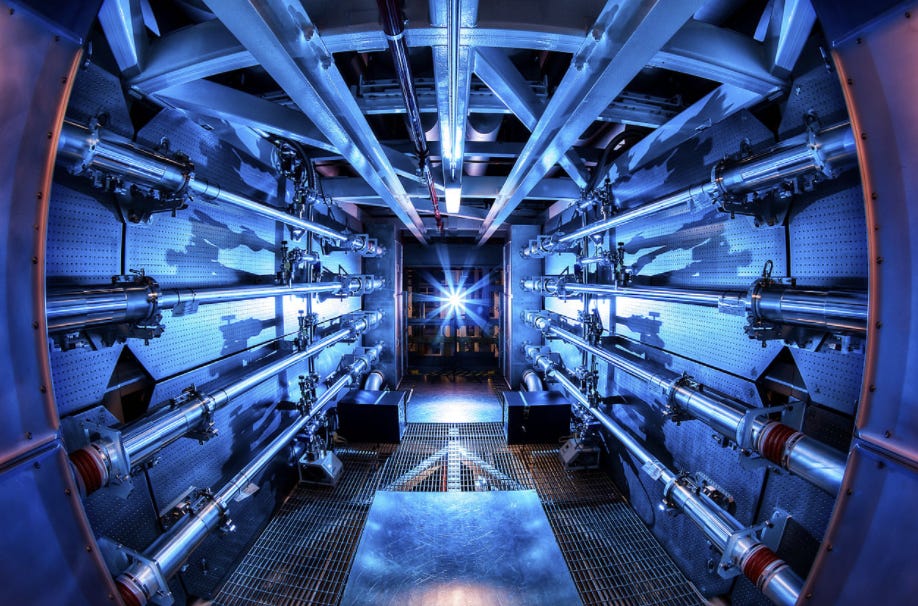
Fusion power is currently speculative. We regularly hear of “big steps toward break-even,” where the power output of the reactor is beginning to match the power input, the power required to turn it on and run it.
This is all hogwash.
Whether a tokamak like JET, or laser-ignited fusion like at Sandia National Laboratory, these facilities are nowhere near actual commercial break-even.
I’ll explain why in a moment, but for those of you that don’t know, fusion nuclear energy is the smashing together of hydrogen to produce helium, just like what happens in one of the deepest layers of most stars, usually near the core. Hydrogen nuclei are positive, and they electromagnetically repel each other REALLY strongly. That said, if you can cram them together close enough despite that intense repulsion, then a different nuclear force takes over, the strong nuclear force. This force is basically the leakage of the attraction of quarks inside protons and neutrons, and it basically has no power at a distance of a dozen or so times the radius of a hydrogen nucleus. But if you get inside that radius, it latches those two hydrogen nuclei together like you wouldn’t believe, sticking 100 times stronger than the electromagnetic repulsion wants to drive them apart.
The only good way to get hydrogen nuclei this close to each other (so far) is with a ton of kinetic (fast pushing) energy. This basically means high temperatures. Temperature is just a measure of the average speed, well, momentum, of particles of something. So you have to get even heavy hydrogen (with extra neutrons in it) up to a few hundred million degrees in order to get a decent amount of fusion happening. You can’t really heat something up that high continuously because if it touches the wall of your furnace, it melts them. Nothing on earth stays solid or even liquid at 10,000 degrees, not to mention 100 million degrees.
So you either put it in a toroidal magnetic bottle and heat it up electromagnetically (a tokomak) or you make a little target of hydrogen in a gold tablet and blast it all at once with a ton of lasers (laser-ignited).
Recent claims of getting to 70% or so of break-even leave out some very key information which turns those claims much less impressive:
a. The processes that heat up the hydrogen (that put energy into the system) are far, far from 100% efficient. In the case of the tokamak, the input efficiency is on the order of 5%. For the laser-ignition facility, the laser efficiency is around 1%.
b. The maximum likely efficiency of converting the resultant heat from fusion is on the order of 60%, with an absolute maximum efficiency of around 68%.
But the claims made are the energy in that get to the atoms (not counting inefficiencies of the heating processes) and the total heat energy from the fusion (not counting the inefficiencies of converting that heat to electricity).
Thus, fusion needs to become much more efficient in order to be commercially viable.
There is also the matter of cost. Current experiments such as ITER and the Livermore experiment are incredibly expensive, 10-50 times more expensive than a typical large power plant. Presumably those costs could go down with engineering around a finalized working design.
But one should be very realistic about the future of fusion power. Yes, there is unlimited fuel and very little radioactive waste. Yes, there are effectively no other pollutants or carbon emissions. It would also be continuously available power, day or night.
Prediction: Commercial nuclear fusion power is not close. And even when it does become close, it will have to compete with another fusion-powered choice.
Wind Power
I bet you thought I was going to say solar. But the Earth’s winds exist because of the uneven heating of the Earth by the Sun, which is of course powered by nuclear fusion.
Wind turbines are remarkable in their construction. These fiberglass and steel behemoths capture up to 14 megawatts of wind energy and convert it into rotation, which drives an electrical generator at their hub nacelle (the cylindrical structure behind the blades). Because that electricity needs to be at a certain frequency (60Hz for some places, 50 Hz for others), there is a gearbox that converts the slow 0.3-1.3 rotations per second into the 60 or 50 rotations per second needed by the generator.
The amount of energy that can be captured by a single wind turbine is proportional to the area swept by the three blades as they make their giant circles. This is why blade sizes (and thus tower heights) keep getting larger and larger. The latest version have blades that extend over 350 feet, making a circle that is 720 feet across. The total height of the tower plus blade is 850 feet tall. Keep in mind that this skyscraper-sized monstrosity has moving blades whose tips go hundreds of miles per hour continuously. Producing 14 megawatts of power at its peak, it holds the world record.
Wind turbines eat no fuel and produce power whenever the wind is blowing hard enough. Most place don’t have sufficiently consistent winds, but many places do. This is why there are now well more than 300,000 wind turbines in the world.
That number will keep growing, and along with it a big maintenance problem.
The cost of maintenance is a big consideration for any energy infrastructure. Wind turbines are particularly difficult. In order to inspect or service a wind turbine, a technician must climb to the top of the wind turbine. Most of these do not have elevators, though more and more have electric winches. Still, it is a difficult and increasingly long journey to the top of these wind turbines to inspect the nacelle and fix anything that might go wrong.
While most wind turbines cost around $2-4 million, their cost of ownership is around $45,000 per year, or about $22,000 per MW per year. For an expected 20 year lifetime, this comes out to almost a million dollars per wind turbine, or around 1/3 of its initial cost. For solar farms, this cost of ownership is somewhat less, typically about $17,000 per MW per year. This is just a little bit more than the typical natural gas cost of $14,000 per MW per year. Nuclear is way, way worse, close to $100,000 per MW per year. In the industry, this is usually categorized as “O&M costs,” or Operations and Maintenance costs. This is a very important number for utilities, as it is the main ongoing cost that they have control of, and it largely determines their profitability. See the O&M costs column in this table from the Energy Information Agency. It’s in $/kW/yr, so to get to the same units I am using above ($/MW/yr), you need to multiply by 1,000.
So wind turbines aren’t great, but they aren’t awful either in this very important metric. Whenever you evaluate a new energy technology, you need to consider the O&M costs.
I would be remiss if I didn’t mention a very important human factor associated with wind turbines: they dwarf humanity. When they are installed far away from towns and cities in the middle of nowhere, or far offshore, this is no problem. But when they are installed near human habitations and roads, they feel oppressive to many people. There is a type of architecture invented to engender the overpowering might of the state, to subconsciously impress might into the minds of the populace. It is called Brutalism, and it is now banned in use for new federal buildings in the United States, which I love. I am not saying that wind turbines are intended to oppress the human spirit, but I fear that they sort of do. They are so large and moving. Some of them make a continuous sound that can be heard for miles. I don’t see a lot of people talking about this, but I think it is something that should be considered, and wind turbines should be kept mostly out of sight.
One more thing: Yes, they kill some birds. But building windows kill about 10,000 times as many birds as wind turbines. So wind turbines are not a proportional/significant contributor to overall bird deaths.
Prediction: Wind turbine installations will continue to increase throughout the century, though their initial and ongoing O&M costs are not as good as for solar panels, and solar may grow much faster. There will be movements to keep wind turbines away from human dwelling places. Still, wind turbines will generally be a consistently growing renewable energy success story.
Solar Panels
They don’t move much. They don’t need fuel. Then don’t emit anything. They require very little maintenance. But they don’t work under clouds or at night, and they only grab around 27% of the sun’s energy.
Solar panels are a fantastic choice. They do take up significant space, but the Earth has a lot of free space. For solar panels, you need around 10 acres per MW. By comparison, you need about 60 acres per MW for wind3. Natural gas plants do about 0.04 acre per MW.
Everyone knows that the main issues for solar panels are cost and intermittency. The cost of panels is now typically less than half the total solar farm cost, with overall prices continuing to fall fairly dramatically. These costs will likely start to level out the second half of this decade, but already solar farms are usually profitable if the local (region-level) grid can handle the intermittency.
For grids with lots of natural gas turbines (which help with the solar power swings), solar panels work great. But those natural gas plants can never fully retire. Solar panels need natural gas plants in order to stay relevant (and one could perhaps argue the converse).
This is where power storage comes in. If power storage can become cheap enough and available in large enough quantities, then solar panels can become the main source of power for large swaths of the globe (but not all).
How much storage is needed? Enough to run the grid on a battery through any perceived length of cloud cover or darkness. People will never again accept power brownouts and power rationing in most countries where they have become accustomed to it. But most of us live in areas that will have at least a week of straight cloud cover sometimes. Based on this, we either need to have enough batteries to last cities for over a week, or we have to upgrade our grids to distribute power over areas two to 10 times larger than we currently do.
Prediction: Solar will be king, but only if we can get enough batteries deployed and/or keep enough natural gas plants running to take up the slack on cloudy days and long nights.
In Part Two of this article next week, we will discuss energy storage technologies and economics, as well as more extreme power transmission technologies.
But really quickly, I wanted to note that in our journey today I snuck in a ton of very deep industry concepts in each section:
In Hydropower, I taught you about how turbines and generators work.
In Tidal Power, I taught you about how energy storage makes lots of energy tech feasible.
In Geothermal, I taught you how to think about deriving capital costs from basic physics principles.
In Nuclear Power, I taught you about base load and why plant load flexibility is important.
In Coal, I taught you about unpaid externalities.
In Natural Gas, I taught you about the value of peaker plants and daily power demand cycles.
In Fusion Power, I taught you about total plant efficiency, because most power plants convert heat to electricity in one way or another.
In Wind, I taught you about the importance of considering O&M (ongoing) costs.
In Solar, I taught you how much storage is needed to enable the use of intermittent power sources.
Energy is the key to future human progress. The better we understand it, in all its intricate details, the better off our society will be.
Yes, scrubber technology may advance, but as we will see there is a physics and cost limit to how much work scrubbers can economically perform.
Not In My Back Yard.
Each 2MW turbine only needs around an acre, but you have to space them out quite a bit. So averaged over an entire wind turbine farm, it comes out to about 60 acres per MW, or 120 acres per turbine.
I love reading these man, you make it so exciting and fun to learn.
This paper must have been quite an undertaking Joshua, and I thank you for investing so much of your time in it. Without reservation, a great job covering developing technologies.
I'm reminded of a book that we covered in my early Engineering program in '85-'86 "Energy: A Crisis, a Dilemma or Just Another Problem?", by J. Doolittle, Matrix Pub; Subsequent edition (January 1, 1983), ISBN-13: 978-0916460334. If you don't already have a copy, it would be a great addition to your bookshelf for comparison of technology progression. You may be able to find a copy on Alibris.com.
Kind Regards,
Terry Terezakis